For rotary AC motors, asynchronous (induction) motors are more common than synchronous motors. But when it comes to linear motion, linear synchronous motors, abbreviated LSMs, are more common.
The vast majority of LSMs I’ve seen have permanent magnets, so this article focuses exclusively on permanent magnet LSMs.
Structure of Linear Synchronous Motors
In general, linear motors are constructed by unrolling rotary motors. Figure 1 shows what I’m talking about. The top portion presents a rotary synchronous motor and the bottom portion shows what the unrolled LSM looks like. Like rotary synchronous motors, LSMs receive AC power, usually split into three phases. But the terms used to describe the elements of an LSM are different:
- The moving element is called the forcer, not the rotor.
- The stationary element is called the rail or track, not the stator.
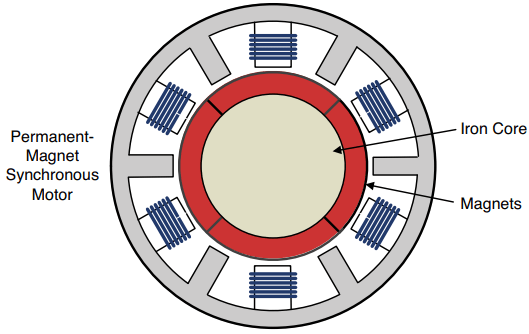
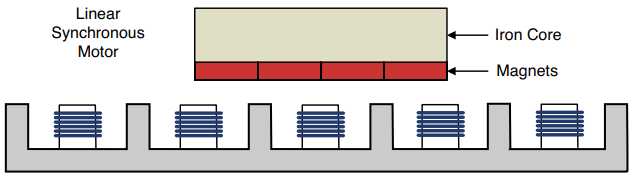
In the figure, the magnets are on the forcer and the windings are on the track. This is called a long stator design. In a short stator design, the windings are on the forcer and the magnets are on the track. The following discussion presents three short stator motors: iron-core LSMs, ironless LSMs, and slotless LSMs. But first, I’d like to explain how synchronous speed is computed for linear motors.
Linear Synchronous Speed
For a linear synchronous motor, if the input power has frequency f, the time of the rotation is 1/f. The situation is similar here. One cycle of AC power corresponds to a change in the magnetic field that travels from Winding A back to Winding A. As shown in Figure 2, this distance equals 2τ.
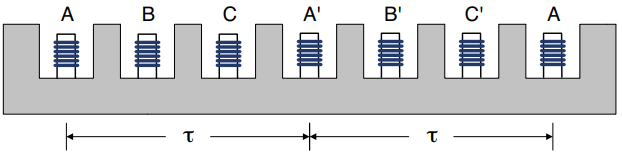
If the input power has frequency f, the time required to travel across a cycle is 1/f. Therefore, the motor’s linear synchronous speed is given by the following equation:
vs = distance/time = 2τ/(1/f) = 2τf
The operating speed of an LSM equals this value. For linear motors, it’s given in units of meters/second instead of revolutions per minute. Also, the equation doesn’t depend on the number of poles. This is because an increase in the number of poles produces a corresponding increase in τ.
Iron-Core Linear Synchronous Motors
An iron-core LSM is essentially similar to the linear motor shown in Figure 1, but the windings are on the forcer and the magnets are on the rail. Figure 3 shows what this looks like.
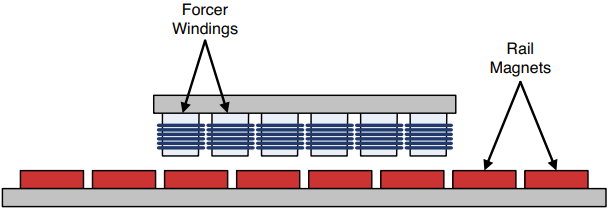
This is referred to as an iron-core LSM because the forcer’s windings are wrapped around iron cores. This increases the amount of force the motor can deliver, which increases the maximum load that it can push, pull, or carry.
One drawback of iron-core LSMs involves the forcer’s weight and the iron’s attraction to the rail magnets. Because of the weight and magnetic attraction, the motor designer must take careful steps to ensure that the forcer doesn’t come in contact with the rail. This usually involves a sliding mechanism that keeps the forcer at least 0.75 mm away.
Because of the iron’s attraction to the rail magnets, the forcer may be reluctant to pass from one magnet to the next. This phenomenon, called cogging, produces a jerky or rippling motion as the forcer moves. It can be reduced by moving the forcer at high speed and orienting the magnets at an angle.
Ironless Linear Synchronous Motors
As its name implies, an ironless LSM doesn’t have iron cores in its forcer. The windings are wrapped around air, and for this reason, ironless LSMs are commonly referred to as air core motors.
Without the weight and magnetic attraction associated with iron, the forcer can move smoothly across the track, without cogging or attraction to the rail’s magnets.
The primary drawback of ironless LSMs is that the forcer can only exert a small amount of force. For this reason, iron-core LSMs are used in applications involving high loads and ironless LSMs should be used when precision and speed control are more important.
To increase the force that can be exerted, the track of an ironless LSM has two rows of permanent magnets. These magnets surround the forcer, which is mounted vertically. Figure 4 depicts a cross-section of a typical ironless LSM.
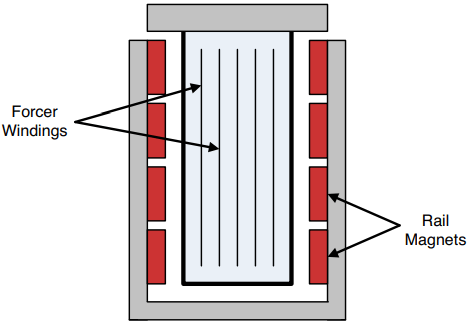
Another disadvantage of using ironless LSMs is cost. Permanent magnets are expensive, especially the rare-earth magnets commonly used in linear motors.
Ironless LSMs have two rows of magnets, and this makes them significantly more expensive than iron core LSMs. The expense increases as the length of the track increases. The enclosed structure of the ironless LSM makes it difficult to dissipate heat. For this reason, ironless motors can’t be run at the same current levels as an iron-core motor.
In addition, it’s common to have heat sensors on the forcer to ensure that the motor isn’t damaged during operation.
Slotless Linear Synchronous Motors
A slotless LSM serves as a compromise between an iron-core and an ironless LSM. Like an iron-core motor, its track has a single row of magnets and the forcer is positioned on top of the track. Like an ironless motor, the windings are wrapped around air. Figure 5 gives an idea of what this looks like.
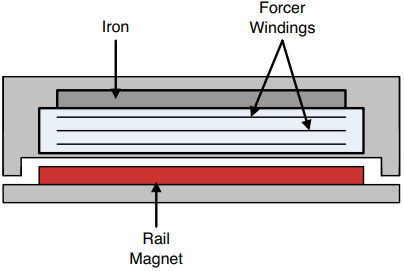
Slotless motors can’t support the same high loads as iron-core motors, but because of the iron backing, they can exert more force than ironless motors. They also have better heat dissipation than ironless motors because the forcer travels on top of the track. Further, the track has only one row of magnets, which makes it less expensive than motors with two rows of magnets.
The main drawback of slotless LSMs involves efficiency. Slotless motors don’t have iron-core windings or multiple rows of magnets. Therefore, to obtain the same degree of motion, a slotless motor requires more power than a comparable iron-core or ironless motor.
Case Study: Transrapid Maglev System
The term Transrapid refers to three distinct entities:
- A set of maglev (magnetic levitation) trains built between 1970 and 2007.
- The company that constructed the Transrapid trains (called Transrapid International—a joint venture of ThyssenKrupp AG and Siemens AG).
- The design technology used to build the Transrapid trains, which refers back to a German patent in 1934.
Transrapid International has constructed nine train lines, and the most recent is the Transrapid SMT (Shanghai Maglev Train). This has many advantages over conventional trains, including greater speed, silent operation, and a lack of moving parts. It’s also the fastest commercial train in current operation, carrying up to 574 passengers at speeds up to 268 miles per hour.
All of the Transrapid trains rely on iron-core linear synchronous motors for propulsion. Unlike the other LSMs discussed so far, the windings are located on the rail, which is commonly referred to as the guideway in maglev systems. Magnets are located on the underside of the train, and Figure 6 shows what the overall design looks like.
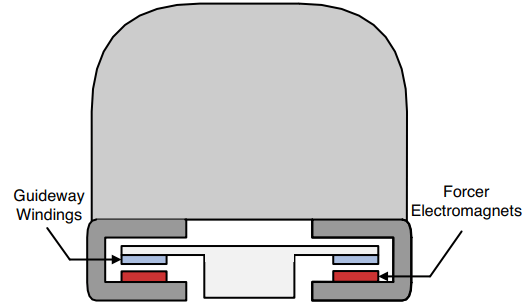
As shown in the figure, the train wraps around the bottom of the guideway, where the guideway’s windings interact with the train’s magnets. These windings receive three-phase AC power, and the only portion of the guideway that receives power is the region directly under the train. The train can be brought to a halt by reversing the current in the windings.
Beneath the guideway’s windings, the forcer magnets are DC-powered electromagnets, not permanent magnets. This is necessary because these magnets serve double-duty. In addition to their role in propulsion, they attract the train to the guideway, thereby making levitation possible. The power to these electromagnets is precisely controlled to keep the train at a distance of about 1 cm from the guideway.
Linear Induction Motors
Linear induction motors, or LIMs, have a great deal in common with LSMs and can be used in the same applications. Structurally speaking, the main difference is that LIMs don’t have permanent magnets. Instead, motion is made possible by a conductor that receives induced voltage from the traveling magnetic field.
Structure and Operation: The induction motors, which have two fundamental properties:
- AC power enters windings in the stator, which combine to produce a rotating magnetic field.
- The stator’s changing field induces a voltage and current in the rotor’s conductors, which results in a force that turns the rotor.
A linear induction motor is constructed by unrolling the elements of a rotary induction motor. That is, the stator of a linear motor consists of windings arranged in a line. Similarly, the rotor consists of a linear conductor facing the stator. Figure 7 shows what this looks like.
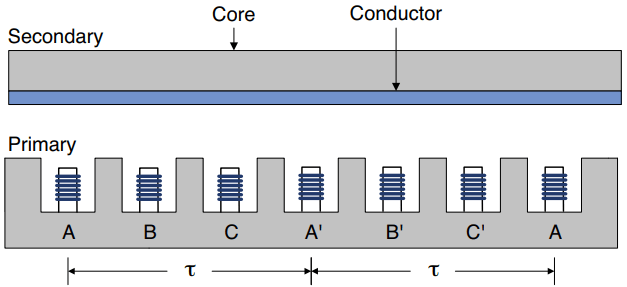
In this figure, the positions of the two elements can be reversed so that the windings move on top of the conductor. Because both elements can move, LIMs don’t use the terms rotor and stator. Instead, the element that creates the changing magnetic field is called the primary and the element that receives induced voltage is called the secondary .
Linear induction motors are polyphase AC motors. In this case, the secondary receives three-phase power and directs the current to its A/A’, B/B’, and C/C’ windings. The changing field in the primary induces a voltage in the secondary’s conductor. Voltage produces a current, and when a current-carrying conductor enters a changing magnetic field, the result is force.
A LIM’s speed, denoted as v, is always less than its linear synchronous speed. The relationship between the two is called the slip, which can be computed with the following equation:
s = (vs –v)/vs
Note that slip is commonly expressed as a percentage.
The LINIMO Train Line
At the 2005 World Expo, Japan demonstrated one of the first commercially available maglev train lines: the Linear Motor line, or the LINIMO. This relies on a linear induction motor to transport passengers from Fujigaoka to Yagusa.
In 2004, researchers at the Tokyo University of Science released a report that provides information about the LINIMO’s construction and operation. One notable point is that the motor makes use of a short stator design. That is, the primary is part of the train, and is positioned above the secondary. This differs from the long stator design employed by the Transrapid discussed earlier.
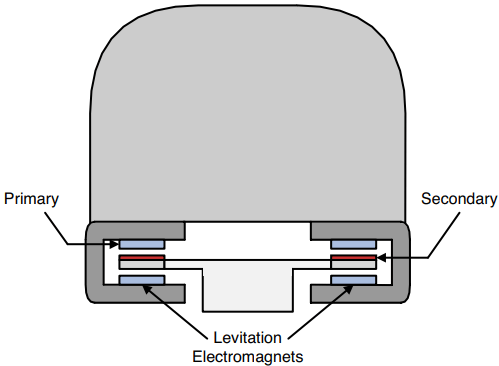
Figure 8 depicts a cross-section of the LINIMO train and platform. As shown, the overall structure is similar to that of the Transrapid.
Relying on induced voltage in the secondary, the LINIMO commonly travels around 60 miles per hour. This is significantly slower than the Transrapid, which travels around 268 miles per hour. Also, the LINIMO moves with less force. The train line must be shut down when wind speeds exceed 25 m/s.
The primary receives three-phase AC power and distributes it to a series of windings. Figure 9 shows how a portion of these windings are arranged on one side of the primary.
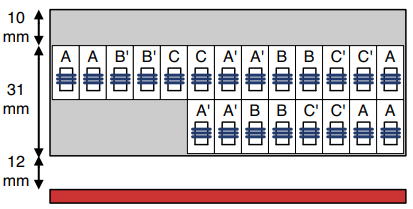
Each winding in the primary has three turns of wire and each receives 300 A. They’re stacked into two tiers, with the windings of the second tier staggered relative to those in the first. As shown in the figure, the windings are only tens of millimeters in size. Personally, I find it incredible that such small electromagnets can move a large train.
The LINIMO’s secondary is simpler in structure. A layer of aluminum is mounted to the top of an iron extension to the platform. This conductive layer is referred to as the reaction plate . Below the primary, the train has electromagnets that keep the train at the proper distance from the platform.
These are called levitation magnets or lift-guide magnets (LGMs). These electromagnets receive DC power, and as more current is applied, the attractive force between the magnets and the platform increases. A proximity sensor is used to ensure that the gap is kept to 8 mm.